Hydrology Basics and the Hydrologic Cycle
ID
BSE-191P
This fact sheet presents and explains some common concepts in hydrology and the hydrologic cycle. The science or study of hydrology focuses on the distribution, occurrence, circulation, and properties of water in the environment. At its most basic level, hydrology is often defined as the study of water; however, basic concepts in hydrology quickly become complex as they are applied to real-world systems to understand and predict what is occurring. This fact sheet summarizes these complex relationships and highlights important applications of hydrologic concepts in agroecosystems, including conservation of soil water in the vadose zone1 to support crop production and water quality.
There are approximately 340,000,000 cubic miles of water on Earth. If all of this water were poured into a sphere, it would be nearly 900 miles in diameter. The vast majority of Earth’s water is salt water in the oceans. Only 2.5 percent of the water on Earth is fresh water, and an even smaller portion of that (0.034 percent) is fresh water readily available to humans. Table 1 shows the locations and relative proportions of water on Earth. Where water is located and its accessibility are important drivers of human habitation, distribution, and success, and they are particularly critical to agricultural production.
One of the most fundamental properties of water is that it is neither created nor destroyed. That is, there is the same amount of water on Earth today as there was millions of years ago; water just changes phases — from liquid to solid to gas. However, while the mass of water is conserved, water quality is not, and degradation of water quality effectively reduces availability of accessible waters for domestic, industrial, or agricultural uses.
Water source | Water volume (cubic miles) | Percentage of fresh water | Percentage of total water |
---|---|---|---|
Oceans | 321,000,000 | – | 96.5000 |
Glaciers and permanent snow | 5,773,000 | 68.700 | 1.7400 |
Groundwater | 5,614,000 | – | 1.6900 |
Saline | 3,088,000 | – | 0.9300 |
Fresh | 2,526,000 | 30.100 | 0.7600 |
Ground ice and permafrost | 71,970 | 0.860 | 0.0220 |
Lakes | 42,320 | – | 0.0130 |
Fresh | 21,830 | 0.260 | 0.0070 |
Saline | 20,490 | – | 0.0060 |
Soil moisture | 3,959 | 0.050 | 0.0010 |
Atmosphere | 3,095 | 0.040 | 0.0010 |
Swamp water | 2,752 | 0.030 | 0.0008 |
Rivers | 509 | 0.006 | 0.0002 |
Biological water (plants and animals) | 269 | 0.003 | 0.0001 |
Adapted from Shiklomanov (1993).
Two types of pollution impact water quality: (1) point source pollution, which is direct discharge into the environment such as from wastewater treatment plants; and (2) nonpoint source pollution, which is composed of diffuse inputs such as urban stormwater or agricultural runoff. Transportation of pollutants involves processes occurring both above and below the ground surface. Pollutants typically associated with surface runoff include excess sediment and phosphorus, while groundwater can transport nitrogen and discharge contaminated waters to streams and rivers as baseflow. Because transport of nonpoint source pollution is mainly driven by the movement of water, an understanding of hydrology and the hydrologic cycle is critical to managing our water resources to support human needs.
The water consumption of humans for domestic use ranges from 3 to 5 gallons per person per day in developing nations to 150 gallons per person per day in the U.S. If industrial and agricultural water consumption is included in the calculation, the average per capita fresh water use in the U.S. is approximately 1,340 gallons per day (WBCSD 2009). Ensuring adequate water supply for these uses requires that three important characteristics of water are sufficient: accessibility, quantity, and quality.
Components of the Hydrologic Cycle
The hydrologic cycle is composed of two phases, the first of which is the atmospheric phase, which describes water movement as gas (water vapor) and liquid/solid (rain and snow) in the atmosphere. The second phase is the terrestrial phase, which describes water movement in, over, and through the Earth. The terrestrial phase is often broken down into the surface water phase (runoff, streamflow) and the groundwater phase (infiltration, percolation, aquifer recharge). The following sections further define the individual components of the hydrologic cycle. Refer to figure 1 for a graphical interpretation of how the various components interact.
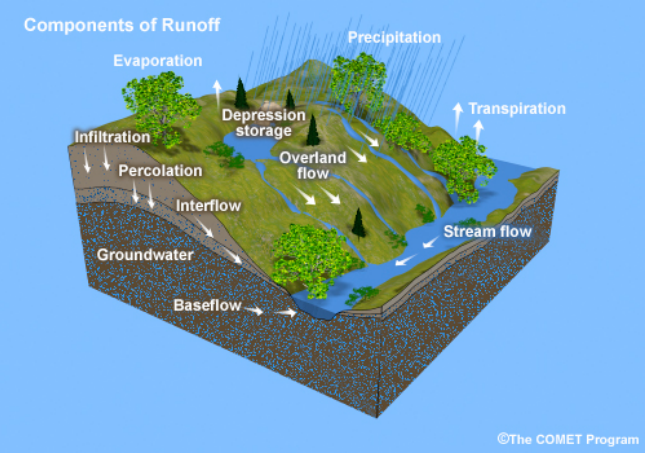
Precipitation
Precipitation is any type of condensation of atmospheric water vapor that falls under gravity and includes rain, snow, sleet, hail, and fog. The amounts and types of precipitation affect soil development, vegetation growth, and the generation of runoff, which transports soils, nutrients, and pollutants. Water that evaporates (or sublimates) from the Earth’s surface is stored as water vapor in the atmosphere before returning to the Earth’s surface as precipitation. As rain falls from the atmosphere, some is caught by vegetation (trees, grass, crops), and this is called “interception.” A portion of intercepted rainfall is evaporated back to the atmosphere from the plant surfaces and never reaches the ground. The rainfall that does reach the soil surface is referred to as net rainfall.
Annual precipitation in Virginia averages 40 inches statewide and ranges from 38 inches in the western mountains to about 45 inches on the eastern Coastal Plain (Southeast Regional Climate Center 2012). Important aspects of precipitation include the annual amount and type (rainfall versus snowfall), which has important consequences for plant water availability, soil moisture, and groundwater recharge. Raindrop size and rainfall intensity (quantity of precipitation per unit time) affect soil erosion; storms that produce higher rainfall intensity and larger raindrop size generally produce more soil erosion. The duration and frequency of storms affect conditions such as flooding and drought. For instance, if 50 percent of the annual rainfall total comes in one event, there is a higher likelihood of flooding during that period and of subsequent drought in the remaining period. The seasonal distribution of precipitation is also important (table 2; fig. 2). Ideally, adequate but not excessive rainfall occurs throughout the agronomic growing season to ensure crop growth, with the remaining precipitation distributed relatively evenly throughout the year. Due to reduced plant uptake, precipitation that occurs outside the growing season has a greater opportunity to infiltrate the soil and recharge groundwater.
Month | Eastern VA | Central VA | Southern VA | Western VA mountains | Northern VA |
---|---|---|---|---|---|
Jan | 3.5 | 3.1 | 3.1 | 2.8 | 3.0 |
Feb | 3.4 | 2.9 | 3.4 | 2.9 | 2.6 |
Mar | 3.8 | 3.8 | 3.8 | 3.8 | 3.4 |
Apr | 3.1 | 3.0 | 3.3 | 2.8 | 3.4 |
May | 3.6 | 3.7 | 3.6 | 3.6 | 4.1 |
Jun | 3.6 | 3.7 | 3.8 | 3.6 | 4.2 |
Jul | 4.7 | 4.9 | 3.6 | 3.6 | 3.8 |
Aug | 4.7 | 4.9 | 4.2 | 3.9 | 4.1 |
Sep | 4.4 | 3.9 | 4.2 | 3.1 | 3.5 |
Oct | 2.8 | 3.3 | 3.3 | 3.2 | 3.1 |
Nov | 2.9 | 3.3 | 2.7 | 2.7 | 3.0 |
Dec | 3.1 | 3.3 | 3.3 | 2.9 | 3.0 |
Total | 43.6 | 43.6 | 42.2 | 39.0 | 41.1 |
Source: Data from Southeast Regional Climate Center (2012).
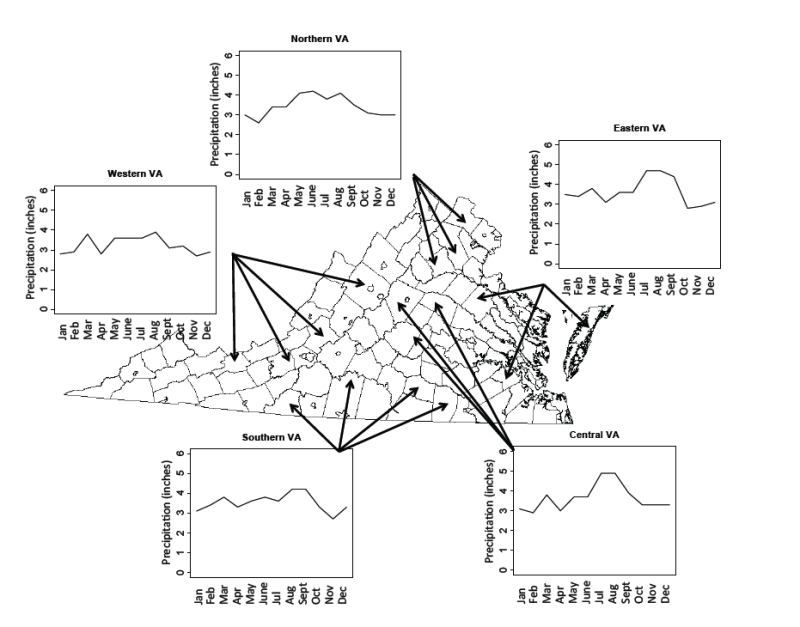
Evapotranspiration (evaporation + transpiration)
Evapotranspiration is the combined effect of evaporation (movement of water directly into the atmosphere as water vapor from a surface, such as the soil or a water body) and transpiration (the process by which plants carry water from the soil into leaves, where it is released to the atmosphere as water vapor). Due to the difficulty in separating the processes of evaporation and transpiration, the two processes are generally considered together and referred to as “ET.” This term includes the water that evaporates directly from soil, water, and plant surfaces and the water that is pulled from the soil by plant transpiration. As much as 75 percent of the water that enters the soil can be returned to the atmosphere through ET. High wind, solar radiation, and heat can greatly increase the ET rate, whereas a high water vapor percentage in the air (high relative humidity) can decrease the potential for ET.
Some producers rely on ET estimates made by government agencies such as the National Weather Service or the National Oceanic and Atmospheric Administration to schedule irrigation or harvesting operations. Often what these agencies report for ET is what is referred to as “potential evapotranspiration” (PET), which is a measure of the maximum ET rate for a given set of conditions defined by solar radiation, temperature, humidity, and wind conditions and also assumes that soil moisture is not limiting (i.e., the soil is at field capacity). In actuality, as plants transpire and remove plant-available water from the soil, the soil begins to dry out, and as a result, the evapotranspiration rate begins to decline. This is because the matric potential or suction of the soil increases, meaning that as the water content decreases, the remaining soil water is held more tightly in the soil pores. The real ET rate (in inches per day), or what is actually evapotranspired, is referred to as actual evapotranspiration (AET) and is influenced by all the conditions that control PET, discussed above, plus the actual soil moisture conditions. Therefore, when the soil is saturated, AET is equal to PET, but AET declines as the soil dries (fig. 3).
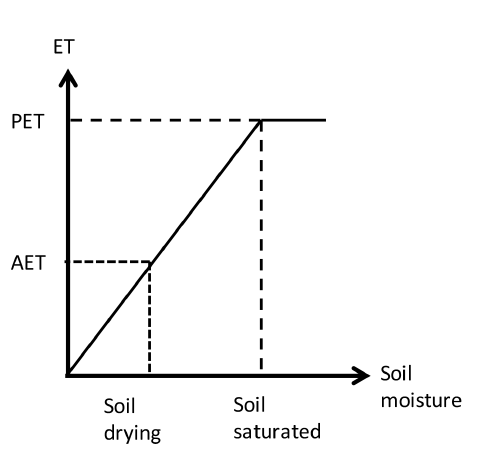
The intensity of ET varies seasonally, with higher rates corresponding to spring and summer months when plants are actively growing and lower rates during the fall and winter months when growth slows and plants become dormant. Plant water requirements and ET drive a seasonal fluctuation of the water table, where the water table is lower during the growing season when ET is high, and the water table height increases as ET decreases during the nongrowing season. Consequently, seasonal fluctuations in ET have a large impact on water availability in agricultural systems.
Infiltration
Once precipitation has reached the soil surface, some of it can infiltrate the soil. Infiltration is the downward entry of water into the soil. The amount of water that infiltrates and how quickly it infiltrates varies widely from place to place and depends on soil properties such as soil moisture content, texture, bulk density, organic matter content, permeability, porosity, and the presence of any restrictive layers in the soil. Permeability is a measure of how fast water flows through the soil. Infiltration and permeability are greater in porous materials, such as sands or gravels, than in clay soils. Porosity is a measure of the total amount of open space or voids in a soil that are capable of retaining water. Water retained in the soil pores is part of the soil storage, a portion of which is available to plants during transpiration. Additionally, plants can utilize water stored in the soil at a later time, providing a buffer capacity for plants between rains. Therefore, soil structure is an important consideration in agroecosystems and can be managed with various tillage practices or soil amendments.
The infiltration rate of any given soil also varies over a rainfall event, usually decreasing significantly as the soil becomes saturated. If rainfall continues long enough, the infiltration rate approaches a constant value comparable to the saturated hydraulic conductivity of a soil (fig. 4). This is an important concept both in hydrology and crop production because saturated hydraulic conductivity is generally related to overall crop productivity. Many soils with low saturated hydraulic conductivity are prone to water logging that may reduce crop yields. When agricultural soils remain saturated too long, the oxygen content in the soil is depleted, causing plant stress. Therefore, strategies including ditch or tile drainage are employed to remove excess water.
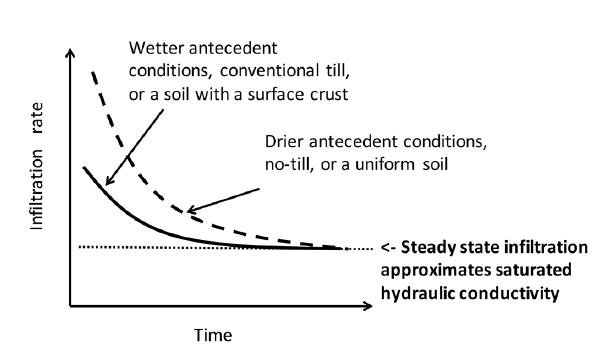
Climatic, land management, and landscape scale factors also influence infiltration. For example, a compacted or frozen soil reduces infiltration. No-till soils will generally have a far greater infiltration capacity than will a compacted soil, where the permeability or hydraulic conductivity is reduced (fig. 4). The presence of vegetation can increase infiltration by promoting macropore formation, increasing permeability, and adding organic carbon to the soil, which reduces the bulk density and promotes biotic activity of soil organisms such as earthworms. Additionally, vegetative cover increases infiltration by protecting the soil’s structure from destruction by raindrops (maintaining porosity) and prevents the formation of soil crusts with reduced permeability. Soil management can also greatly affect infiltration rates. For instance, stable soil aggregation (the structural unit of soil that is bound together, creating porosity) can greatly increase the infiltration rate of fine-textured loams and clays, and tillage — especially under wet conditions — can break down these soil aggregates. Heavy traffic of agricultural equipment on fields can cause soil compaction, further reducing infiltration. Some soils, such as shrink-swell clays, may temporarily have much higher infiltration rates when they are dry than when they are wet due to cracking at the surface and macropore flow, which allows water to move rapidly down into the soil.
Percolation and Groundwater Recharge
Aside from plant uptake, another path that water can take after it enters the soil is percolation. Percolation is the downward movement of water that has infiltrated out of the root zone under the pull of gravity. Generally, percolation is beyond the reach of plant roots. Water that percolates downward through the soil, below the plant root zone toward the underlying geologic formation, is responsible for recharging aquifers.
Groundwater can be a very important source of irrigation water for agriculture. While much of the irrigation in the Mid-Atlantic Region relies on surface water supplies, there is considerable infrastructure in place to utilize groundwater for irrigation. Many producers prefer groundwater because it is of higher quality and less prone to short-term shortages due to the weather.
Groundwater Discharge
Groundwater that has percolated to an aquifer can be stored in that aquifer or can flow out of the aquifer and discharge to a surface water body, such as a stream, river, or lake. This groundwater discharge creates baseflow — the amount of flow in a stream in the absence of additional inputs resulting from storm events — and is an important connection between ground and surface waters.
Groundwater moves from higher elevations to lower elevations or similarly from areas of higher hydraulic pressure to areas of lower hydraulic pressure. This pressure difference between two points is often referred to as hydraulic head gradient. Groundwater movement always follows the downward hydraulic head gradient; with no hydraulic head gradient there is no lateral groundwater movement. Similar to the infiltration rate, the rate of groundwater movement is controlled by the permeability of a geologic material. In areas of steeper terrain or with gravely or sandy geologic material, groundwater movement can be quite rapid, on the order of 10-1,000 feet per day, while low gradient areas or areas with lower-permeability geologic material conduct water less rapidly, at 0-10 feet per day.
Interflow
Interflow is the lateral movement of infiltrated water in the vadose zone and is influenced by soil, geologic, and terrain properties in the surrounding area. As water infiltrates, some of it may reach a layer of soil or rock material that restricts downward movement and causes a perched water table. Restrictive layers can form naturally (fragipan, clay pan, bedrock) or as a result of human activities; an example of which is the creation of a restrictive layer with lower permeability than the bulk soil created during tillage, which is referred to as a plow pan. Once water reaches a restrictive layer and can no longer infiltrate vertically, it becomes perched over this layer and may then move laterally as interflow above this layer if there is sufficient hydraulic head or slope (fig. 5). As interflow moves through the soil, it accumulates as more water infiltrates and can saturate the soil profile, as indicated by the size of the interflow arrows in figure 5. Once the soil saturates either from the incoming interflow volume or from reduced soil transmissivity, interflow can then resurface either as runoff on the soil surface (a process called exfiltration), be directly discharged to a surface water body or, in many areas of Virginia, feed springs or seeps (fig. 5). Soils with high permeability in hilly terrain, particularly those underlain by a restrictive layer, can conduct a significant amount of interflow through the profile compared to low-permeability soils on low slopes, which are prone to generating more surface runoff. In agricultural areas, particularly those in hilly terrain, significant quantities of nutrients (particularly nitrogen) can be transported to streams via interflow.
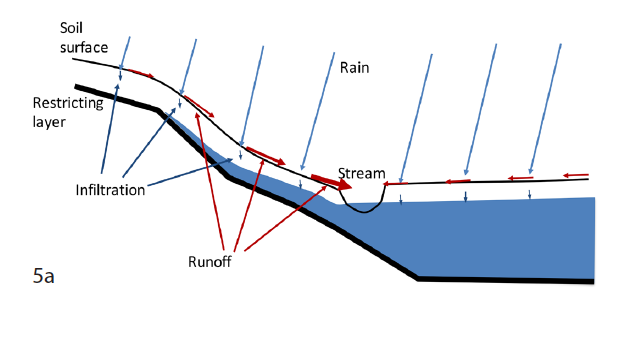
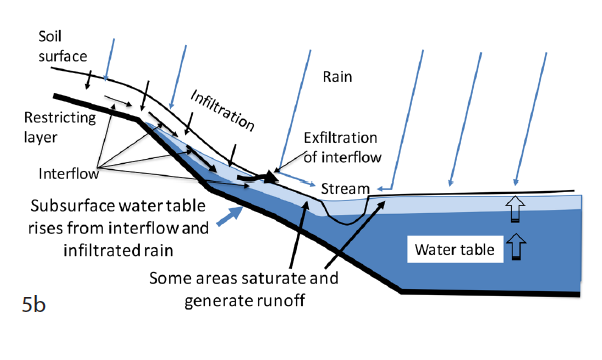
Runoff
There are many ways to describe runoff, but a simple definition is the portion of precipitation falling on an area that is rapidly discharged from the area through stream channels. However, in reality, runoff generation is a complex process controlled by many factors, and there are even different types of runoff generated depending on the conditions. The first type of runoff is called infiltration excess runoff and is the type with which most people are probably familiar. As the name suggests, infiltration excess runoff occurs when the infiltration rate of the soil is less than the rainfall rate (fig. 5). This type of runoff generally occurs during intense thunderstorms or on soils with a low infiltration rate, such as clays or compacted soils, and is more common in urban areas where impervious surfaces dominate. Once the precipitation rate exceeds the infiltration rate of the soil, depressions on the soil surface begin to fill. These surface depressions provide what is called surface storage or depressional storage (fig. 1). When depressional storage is filled and precipitation continues to exceed infiltration, water begins to move down slope as overland flow or in defined channels. In agroecosystems, depressional storage can sometimes be enhanced by tillage. Although tillage can create these depressional storage areas that delay runoff and promote infiltration, often the tillage-induced compaction outweighs this storage, resulting in a net increase in surface runoff.
The second type of runoff generation is called saturation excess runoff, which occurs when the soil profile becomes saturated with water and can store no more precipitation. Thus, any precipitation falling in these areas becomes runoff. Several terrain and soil properties influence saturation excess runoff generation, which is largely governed by topography and soil depth. Soils with shallow restricting layers, areas that drain large upslope contributing areas, or areas where the slope flattens are all prone to generating saturation excess runoff primarily because interflow from upslope areas can no longer be transmitted through the soil profile (fig. 5). Saturation excess runoff is not sensitive to rainfall intensity or soil infiltration rate but depends on where the saturated areas occur in the landscape. This type of runoff generation is far more common in the humid temperate regions, such as the Mid-Atlantic, than infiltration excess runoff, primarily because the region is well- vegetated and has permeable topsoil.
Depending on which type of runoff is generated, there can be varying water quality and production consequences. For instance, sheet and rill erosion are more common during infiltration excess runoff, partially because this type of runoff is associated with high rainfall intensities, while saturation excess type runoff is often responsible for gullying and stream bank failures due to increased pore water pressure from the weight of water in the soil profile, which reduces soil cohesion.
Conclusion
The water on earth is finite, and only a small amount is readily available for human use. This publication presents an overview of the hydrologic cycle and highlights some concepts of importance in agroecosystems. Sound decisions about the use and protection of our water resources require a fundamental understanding of the basic processes of the hydrologic cycle and how water circulates in the land and atmosphere. Understanding the hydrologic cycle is critical because many of the significant environmental and societal problems we face today are related to hydrologic or water issues, including climate change, agricultural productivity and food security, energy, and human health.
Additional Resources
Natural Resources Conservation Service: Erosion Information www.nrcs.usda.gov/wps/portal/nrcs/ main/national/landuse/crops/erosion/
Natural Resources Conservation Service: Soil Textural Triangle
www.nrcs.usda.gov/wps/portal/nrcs/detail/ soils/survey/?cid=nrcs142p2_054167
Southeast Regional Climate Center: Virginia Historical Data - www.sercc.com/climateinfo/historical/historical_va.html
United Nations: Water Use and Availability
www.unwater.org/downloads/Water_facts_and_trends.pdf
U.S. Geological Survey: Groundwater Facts
http://pubs.usgs.gov/circ/circ1186/html/gen_facts.html
Related Virginia Cooperative Extension Publications
Benham, B. 2009. A Glossary of Water-Related Terms. VCE publication 442-758. http://pubs.ext. vt.edu/442/442-758/442-758_pdf.pdf.
Helfrich. L. A., D. L. Weigmann, and R. J. Neves. 2009. Landowner’s Guide to Managing Streams in the Eastern United States. VCE publication 420-141. http://pubs.ext.vt.edu/420/420-141/420-141_pdf.pdf.
Ross, B., T. Younos, and D. L. Weighman. 2009. Virginia Farmstead Assessment System: Site Evaluation: Groundwater, Soils & Geology. VCE publication 422-901. http://pubs.ext. vt.edu/442/442-901/442-901.html.
Glossary of Terms
antecedent soil moisture - The wetness of a soil that results from past rainfall or soil drying events.
aquifer - Saturated fractured rock or other geologic material such as sand or gravel that water can move through. Aquifers serve two primary functions: a storage function, where water is stored temporarily; and a conduit function, where water is translocated from one area to another.
aquifer recharge - The result from the percolation of infiltrated precipitation to subsurface water bearing geologic materials.
baseflow - Typical low-flow discharge in streams in contrast to stormflow that occurs in response to precipitation events; groundwater discharge drives baseflow.
bulk density - The weight of dry soil (the mass of the solid particles) per unit volume of soil.
depressional storage - Water contained in natural depressions in the land surface, such as puddles or furrows.
exfiltration - Process by which infiltrated water in the vadose zone reaches the soil surface and creates runoff or a spring/seep.
field capacity - The amount of water held in the soil 48 hours after a saturating rain. It is what water remains after excess water has drained away and the rate of downward movement has ceased.
fragipan - Cemented or densely packed subsurface soil horizon that prevents water and plant root penetration. Fragipans are common to glaciated regions and often increase perched water table formation and runoff generation because water cannot percolate through the layer.
hydraulic head gradient - The slope of the groundwater surface in an aquifer. The hydraulic head gradient indicates the direction of groundwater flow. Water always flows from higher hydraulic head gradient to lower hydraulic head gradient. All other factors being equal, flow is greater when the hydraulic head gradient is steeper.
infiltration - The downward entry and movement of water through the soil profile.
infiltration excess runoff - Occurs when water enters a soil system faster then the soil can absorb or move it, such as when precipitation exceeds the infiltration capacity of the soil. Also known as “Hortonian flow.”
macropores - Large soil pores that form as a result of biological activity (root channels, worm holes), geological forces (subsurface erosion, desiccation and syneresis cracks, fractures), or mechanical practices (plowing, bores, wells). Macropores are larger than 0.08 mm in diameter and can conduct large quantities of water and agrochemicals deeply into the soil.
matric potential - The sum of adsorptive forces that hold water to individual soil particles and capillary forces that hold water between soil particles. Matric potential is always negative (or zero when the soil is fully saturated) and is strongest in drier soils where the water is tightly bound to soil particles.
organic matter content - Plant and animal residues, cells and tissues, or soil organisms composed of carbon and the substances the organisms synthesize.
perched water table - A vertically confined but laterally unconfined water body that forms over a restricting layer (see “fragipan,” for example) and is isolated from the primary groundwater by one or more layers of unsaturated soil.
percolation - The downward movement of water through saturated soil layers, percolation is responsible for groundwater (aquifer) recharge.
permeability - In soil, a measure of the capacity of the soil to allow fluids to pass through it, expressed as a velocity.
plant-available water - The water available to support plant growth; the difference between field capacity and the permanent wilting point.
porosity - The amount of pore space (air or void space) between soil particles. Infiltration, groundwater movement, and storage occur in these void spaces. Soils with higher clay content have higher overall porosity than sands.
relative humidity - A measure of how wet the air is; defined as the amount of water vapor in the air, expressed as a percentage of the maximum amount of water vapor the air can hold at a given temperature.
restrictive layer - A soil layer of low conductivity (fragipan, bedrock, clay layer) that prevents vertical water movement and fosters the generation of perched water tables, interflow, and runoff.
saturated hydraulic conductivity - A measure of the ability of soil to transmit water when fully saturated; similar to permeability.
saturation excess runoff - Occurs when the soil becomes saturated and any additional precipitation cannot be infiltrated or stored by the soil, causing runoff.
sheet and rill erosion - Sheet erosion is erosion that detaches and removes soil particles more or less evenly from the soil surface and is caused primarily by raindrop impact and uniform overland runoff. Rill erosion forms when overland runoff becomes channelized as it flows down slope and begins to create small erosional channels (rills) up to approximately 8 inches deep.
soil moisture content - The amount of water stored in the soil; primarily influenced by the soil texture and the soil organic matter content. In general, soils with greater silt- and clay-sized particles have higher soil moisture content, all else being equal. Likewise, soils with more organic matter have higher soil moisture content.
solar radiation - The radiation or energy emitted from the sun.
sublimate - The transition of a solid (snow or ice) directly to a gas (water vapor) without passing through the liquid phase.
texture - The relative proportions of sand, silt, and clay that make up a soil. Many soil properties are influenced by texture, including drainage, water-holding capacity, aeration, susceptibility to erosion, organic matter content, cation exchange capacity, pH buffering capacity, and soil tilth. The combined fractions of sand, silt, and clay in a soil determine its textural classification. Sand particles range in size from 0.05 mm to 2.00 mm, silt ranges from 0.002 mm to 0.050 mm, and the clay fraction is made up of particles less than 0.002 mm in diameter. Once the sand, silt, and clay fractions are known, the textural class can be read from the textural triangle. (Soil texture calculator available at www.nrcs.usda.gov/wps/portal/nrcs/detail/soils/ survey/?cid=nrcs142p2_054167.)
transmissivity - The rate at which water can pass through a soil profile at a specific slope; the product of soil depth times soil saturated hydraulic conductivity.
vadose zone - The typically aerated zone above the permanent water table; the zone where the soil water is at atmospheric pressure.
References
COMET Program. 2005. “Surface Water.” Section Four in Understanding the Hydrologic Cycle. Basic Hydrologic Science Course. University Corporation for Atmospheric Research. Boulder, CO: University Corporation for Atmospheric Research.
Shiklomanov, Igor. 1993. “World Fresh Water Resources.” In Water in Crisis: A Guide to the World’s Fresh Water Resources, edited by Peter H. Gleick, 13-24. New York: Oxford University Press.
Southeast Regional Climate Center. 2012. “Historical Climate Summaries for Virginia.” Website. University of North Carolina-Chapel Hill. www.sercc.com/climateinfo/historical/historical_va.html.
WBCSD (World Business Council for Sustainable Development). 2009. Water Facts and Trends. Version 2. Geneva: WBCSD. www.unwater.org/downloads/Water_facts_and_trends.pdf.
Virginia Cooperative Extension materials are available for public use, reprint, or citation without further permission, provided the use includes credit to the author and to Virginia Cooperative Extension, Virginia Tech, and Virginia State University.
Virginia Cooperative Extension is a partnership of Virginia Tech, Virginia State University, the U.S. Department of Agriculture, and local governments. Its programs and employment are open to all, regardless of age, color, disability, sex (including pregnancy), gender, gender identity, gender expression, genetic information, ethnicity or national origin, political affiliation, race, religion, sexual orientation, or military status, or any other basis protected by law.
Publication Date
November 4, 2020