Soil and Soil Water Relationships
ID
BSE-194P
EXPERT REVIEWED
This publication presents and discusses concepts that are fundamental to understanding soil, water, and plant relationships and the soil water balance. Knowledge about soil water relationships can inform the decision-making process in agricultural operations or natural resource management, such as determining what crops to plant, when to plant them, and when various management practices should be scheduled. Understanding these concepts is useful for addressing both agronomic and policy issues related to agricultural water management.
Soil
Soil Composition and Texture
Soils are composed of four components: mineral solids, organic matter solids, water, and air. The solids are made of minerals derived from geologic weathering and organic matter consisting of plant or animal residue as well as living organisms. The empty spaces between the solids, called pores, are occupied by either water or air. The mineral solid fraction of the soil is made up of sand, silt, and clay, the particular ratios of which determine the soil texture. Sand particles range in size from 0.05 to 2.00 mm, silt ranges from 0.002 to 0.050 mm, and the clay fraction is made up of particles smaller than 0.002 mm in diameter. Particles larger than 2.0 mm are referred to as rock fragments and are not considered in determining soil texture, although they can influence both soil structure and soil water relationships. Once the sand, silt, and clay fractions are known, the textural class can be determined using a soil textural triangle (fig. 1). Most land-grant universities and private labs can determine the sand, silt, and clay fractions of soil samples. Soil texture determination is important because many soil properties are influenced by texture, including drainage, water-holding capacity, aeration, susceptibility to erosion, cation exchange capacity, pH buffering capacity, and soil tilth.
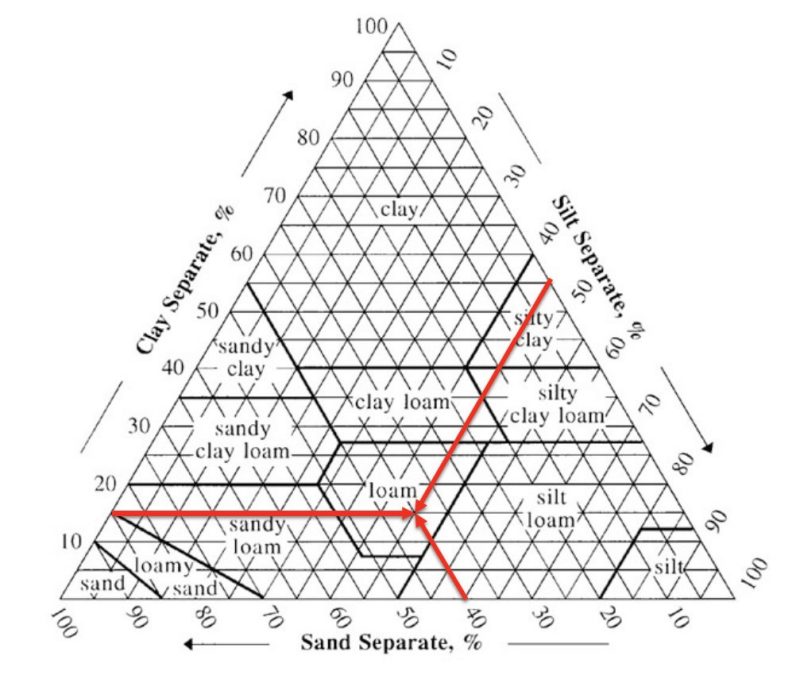
Most soils are composed of about 35-55 percent pore space and 45-65 percent solid material by volume. Between 2 and 5 percent of the solid material is organic-based residue, with the remainder being mineral-based. Agricultural soils, particularly those that are tilled, tend to contain less organic matter compared to forested or undisturbed soils. When organic matter is exposed to soil microorganisms in aerobic conditions, it becomes oxidized and degraded (mineralized). Organic matter is generally viewed as a beneficial soil component because it provides water-holding capacity, slowly releases nutrients for plant growth, and improves soil structure.
Air and water occupy the pore space in a soil, and the proportion of each varies by soil type and over time. After a large precipitation event, the pore space occupied by water will increase, and the air-filled pore space will decrease. During prolonged dry periods, the water-filled pore space will decrease, and the air-filled pore space will increase due to evapotranspiration and percolation of water from the root zone.
Soil Structure
Soil structure refers to how the soil particles are shaped and organized into units of aggregation, also referred to as peds. There are six primary soil structural classes: platy, prismatic, columnar, blocky, single-grained, and granular (fig. 2). Soil structure affects the rate that water and air can move through the soil, root penetration, and the availability of nutrients to plants. Single-grained soils (sands) and massive soils (loams and clays) are called “structureless.”
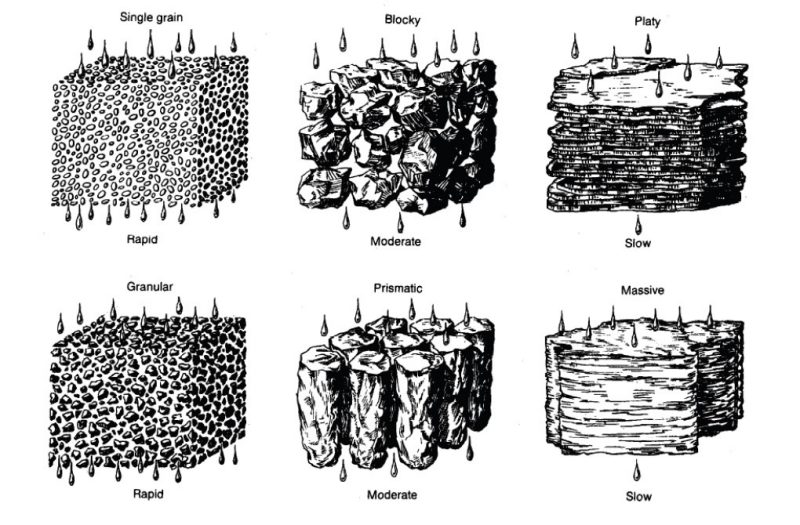
In single-grained soils like sand, water percolates very quickly through the soil, while in massive soils such as dense clays, water movement is very slow. Platy structure impedes the downward movement of water due to the horizontally arranged plates of soil. More favorable water movement characteristics for crop production are generally found in soils that have granular, prismatic, or blocky structure. Well-structured soils are usually more desirable for agricultural production because structured soils can hold and conduct water and gasses and are also able to support load-bearing activities, such as field traffic.
Tillage of some fine-textured soils, particularly when they are wet, can destroy the soil structure, as can irrigation with high sodium (salt) content, which causes dispersion. Biological activity of organisms such as earthworms, mammals, termites, and ants can also change the soil structure by creating large pores from burrowing and deposition of organic matter.
Soil Bulk Density and Porosity
Soil bulk density expresses the ratio of the mass of dry soil to the total volume occupied in the soil. The total volume includes both the solids (mineral and organic) and the pore spaces. Soil bulk density is important because it is an indicator of the soil’s porosity. The porosity of a soil is defined as the volume percentage of pores in a soil the inverse of the volume percentage of solids. Within a soil of similar texture, a compacted soil has lower porosity and thus a greater bulk density, while a loose soil has a greater porosity and a lower bulk density.
Bulk density also provides information about the potential for leaching of agrochemicals, erosion, and crop productivity. Surface runoff and erosion of soil both of which can remove nutrients needed by crops can occur on soils with a high bulk density because water is restricted from moving through the soil and thus ends up as surface runoff and carry away soil and soil-bound nutrients. Soils with lower bulk density, such as sands, can promote vertical leaching of nutrients and reduce their availability to crops. As with soil structure, soil bulk density can be affected by physical management practices, such as tillage, as well as biological activity, such as the tunneling of earthworms. Total soil porosity can be determined for a soil sample using the following equation:
total porosity = 1 - (bulk density/particle density).
Bulk density is generally measured in a soil sample obtained with a core, which is used to extract undisturbed samples from various depths in the soil profile. Particle density is determined similarly to bulk density but is the mass of the dry soil divided by the volume of the solid soil components only, that is, excluding the volume of the pore space. Therefore, particle density will be greater than bulk density and represents the average density of all the solids composing the soil. The particle density of most soils is around 2.65 g/cm3 because quartz has a density of 2.65 g/cm3 and is usually one of the dominant minerals in soils. However, clay has a particle density of 2.83 g/cm3, and organic matter has a particle density of 0.8 g/cm3, so soils with significant amounts of either clay or organic matter particle density will deviate from the 2.65 g/cm3 particle density estimate.
Soil porosity is influenced primarily by soil aggregation, soil texture, root penetration, and other biological activity (burrowing insects, worms, etc.). Coarse-textured or sandy soils tend to be less porous than fine-textured (clayey) soils because although the average size of individual pores is usually larger in coarse (sandy) soils, the smaller pores in the clayey soils are more numerous. Organic matter content increases porosity by enhancing soil aggregation.
Micropores and Macropores
The size of a pore in a soil affects how the pore functions. Macropores, those larger than 0.08 mm, are primarily the pore spaces between aggregates, and they promote free drainage of water (which can transport dissolved nutrients and agrochemicals), aeration, evaporation, and gas exchange in the soil profile. This free drainage, also referred to as “preferential flow” because the water preferentially bypasses the bulk soil, is enhanced by wet soil conditions and lack of soil disturbance such as is often found in no-till or pasture systems. Preferential flow can cause rapid leaching and bypass flow of nutrients and agrochemicals, even those that otherwise could be strongly bound to the soil, thereby potentially reducing the effectiveness of fertilizer and agrochemical applications. Macropores are most prevalent in loamy and well-aggregated soils but can be converted to micropores by compaction through tillage and vehicle traffic.
Micropores, which are 0.08 mm or smaller and occur inside soil aggregates, retain water and agrochemicals and are responsible for capillary water distribution. However, most of the water they contain is not available to plants. Clays promote aggregation but can also be readily compacted. Therefore, clays increase water storage, but not necessarily plant-available water, by providing an abundance of micropores. Clays can, however, increase storage of plant-available water through the formation of stable soil aggregates.
Increasing macroporosity with organic matter amendments, which promotes root penetration and earthworm movement (fig. 3), can improve water infiltration and movement in fine-textured soils. Thus, soil texture and structure, biological activity, and the level of compaction are the main factors that influence the amount and type of pore space in a soil.
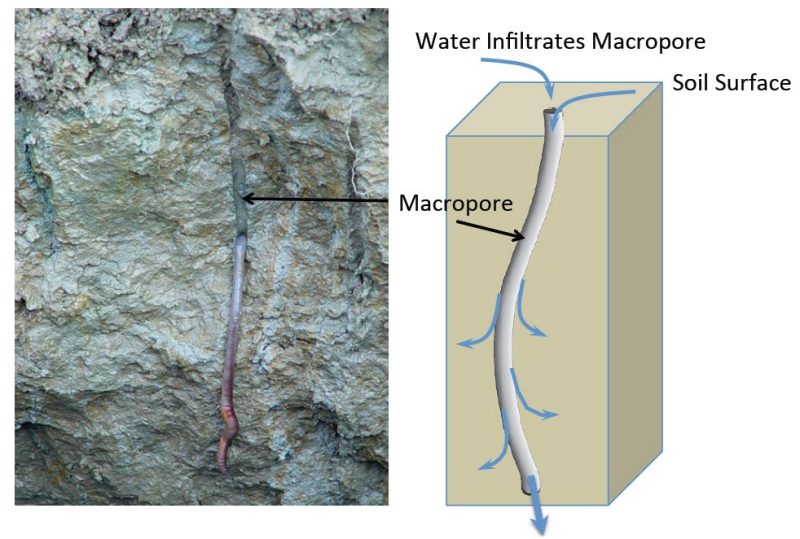
Important Soil Water Relationships
With the background concepts outlined above, we can now develop some basic soil water relationships that are important to consider for agricultural management. The following sections discuss how the soil properties described influence the soil water content, soil water potential, and soil drainage characteristics.
Soil Storage or Soil Water Content
The soil water content is the amount of water held in the soil at any given time and can be expressed as volumetric or gravimetric water content. Volumetric water content is the volume of water per unit volume of dry soil and is the most useful way of expressing water content for developing a water budget, which is discussed in the next section. Gravimetric water content is the mass of water per unit mass of dry soil. The volumetric water content (percent, unitless) is equal to the gravimetric water content (in cm3 per gram) multiplied by the soil’s bulk density (in grams per cm3).
To interpret the soil water content, remember that not all soil water is accessible to plants. The water available to support plant growth is called plant- available water and is the difference between field capacity and the wilting point (fig. 4). Field capacity is the amount of water remaining in the soil profile after 48-72 hours of free drainage following saturated conditions. Field capacity is also defined as one-third of atmospheric tension, that is, water is held in the soil weakly and is easily available for plant uptake. While field capacity is considered to be the upper limit of available water, it should be pointed out that this is not strictly true. Water moving downward in the soil following a saturating event can be effectively used by growing plants. However, because gravitational flow is transitory, this water is generally not considered in calculations to determine the available water capacity of a soil, but it can affect things such as irrigation scheduling. Water that drains freely under gravity is called gravitational water and might or might not be used by plants, depending on environmental conditions.
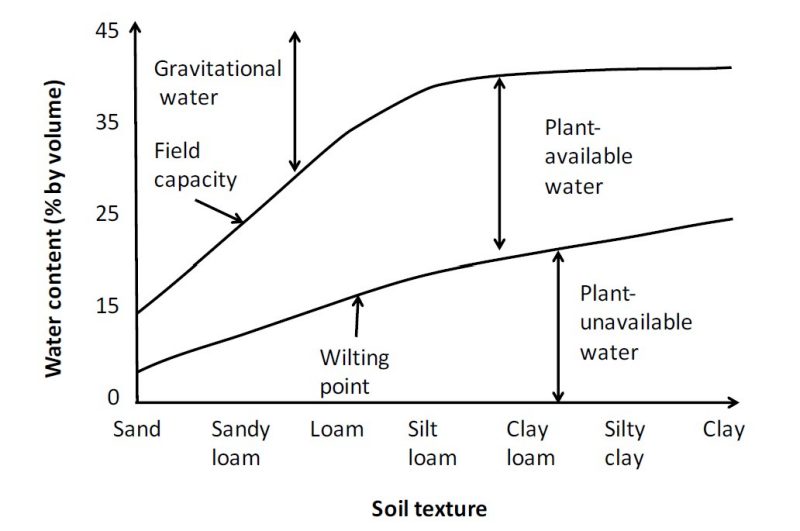
The wilting point is the minimum moisture content of the soil that can support plant growth and below which plants cannot recover. The wilting point is defined as -15 atmospheres, that is, water below this point is held so strongly in the soil that plants cannot access it. Depending on the soil type, there can be very different gravitational, plant-available and unavailable water contents (fig. 4). For example, sands have relatively little plant-available water and a significant portion is gravitational water flowing through macropores, and, as a result, crops grown on sandy soil are more vulnerable to drought. Conversely, clay soils usually have a slightly higher plant-available water content due to enhanced aggregation, but also have a higher unavailable water content because the water is held so tightly in the micropores that the plants cannot access it (fig. 4). Loamy soils have the largest plant-available water content as well as relatively equally sized gravitational and unavailable water contents. For this reason, loam and silt loam soils are highly prized for agricultural production; they drain excess water quickly, have a large plant-available water content, and are not prone to drought conditions (table 1).
Textural class | Wilting point (% moisture) |
Field capacity (% moisture) |
Available water (% moisture) |
---|---|---|---|
Sand | 5 | 12 | 7 |
Sandy loam | 9 | 21 | 12 |
Loam | 16 | 36 | 20 |
Silt loam | 18 | 39 | 21 |
Clay loam | 24 | 39 | 15 |
Silty clay | 24 | 39 | 13 |
Clay | 27 | 39 | 12 |
Soil Water Potential:
How Soil Holds Water
The soil water content discussed above should not be confused with the soil water potential, which is the energy status of the soil water. The soil water potential (also called soil water tension) is the amount of work required to move (extract) water from the soil. Differences in potential energy of water from one point in the soil to another are responsible for the tendency of water to flow within the soil. Water always moves from higher to lower potential (energy status) and not necessarily from higher to lower water content. This concept is important for non-uniform (layered) soils. Adhesion, surface tension, and cohesion at the air/water interface in unsaturated soil pores result in capillary suction and cause the water to be held tightly within the soil. Note that capillary suction increases as pore size decreases, which is why water is held more tightly in micropores present in clay-dominated soils. Total water potential consists of three forces acting on the water:
total water potential = matric potential + gravitational potential + pressure potential.
Gravitational Potential
Gravitational potential is due to the force of gravity pulling on the water. Determination of gravitational potential is independent of soil properties and depends only on the vertical distance between the water elevation and a reference elevation.
Matric Potential
Matric potential is due to the force exerted on the water by the soil, also called tension, and is the combination of adsorptive and capillary forces in the soil (fig. 5). Matric potential is a dynamic property and is essentially zero for a saturated soil and negative at water contents below saturation; matric potential is never positive. The matric forces attract and bind water to the surface of soil particles (fig. 6) and lower its potential energy below that of the bulk water (water not in direct contact with the soil); that is, it takes energy to overcome the matric force and move water from one location to another. Capillarity results from the surface tension of water and its contact with the solid soil particles properties inherent to the molecular structure of water. Strong (very negative) matric potentials bind water to soil particles in very dry soils. Matric potential is generally stronger in finer textured soils than in coarse soils due to greater surface area and smaller pore sizes (fig. 7), and it is for this reason that clayey soils have higher plant- unavailable water content than sands (figs. 5 and 7). This effect is due to the adhesive and cohesive forces at the soil-water interface. As an example, in figure 5 the smaller tube exhibits greater matric potential, a combination of water molecules adsorbing to each other and to the walls of the tube. Figure 7 illustrates this concept in soils. The use of moisture sensors in a soil provides information about the soil matric potential, which can then be used to estimate the soil water content of a soil. For example, in figure 7 a loam with a matric potential of −110 feet is at or near the wilting point and has a water content near 30 percent.
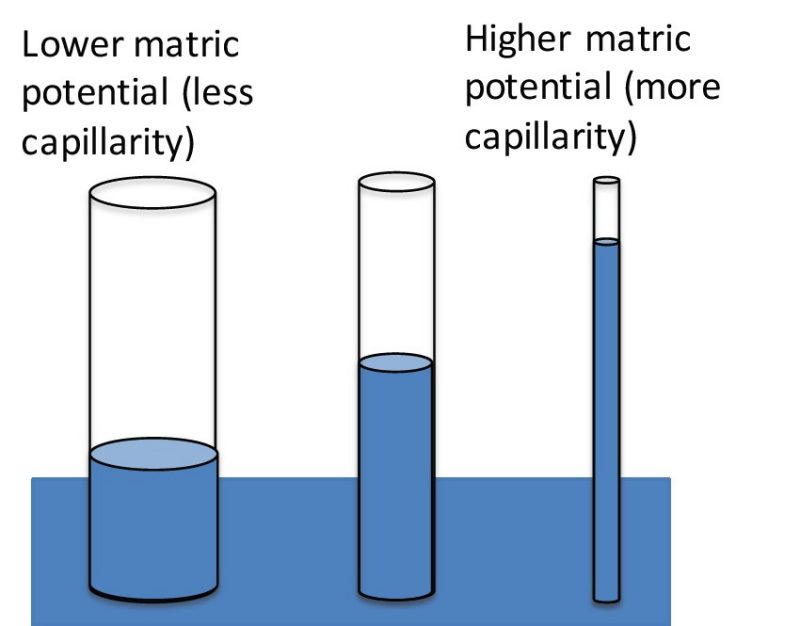
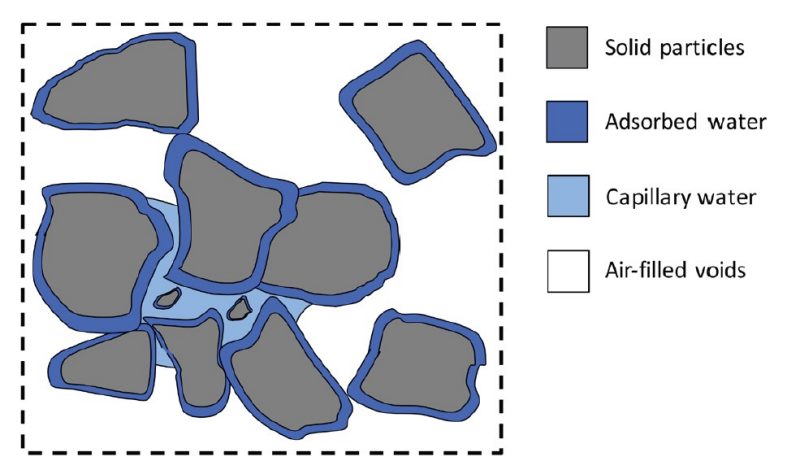
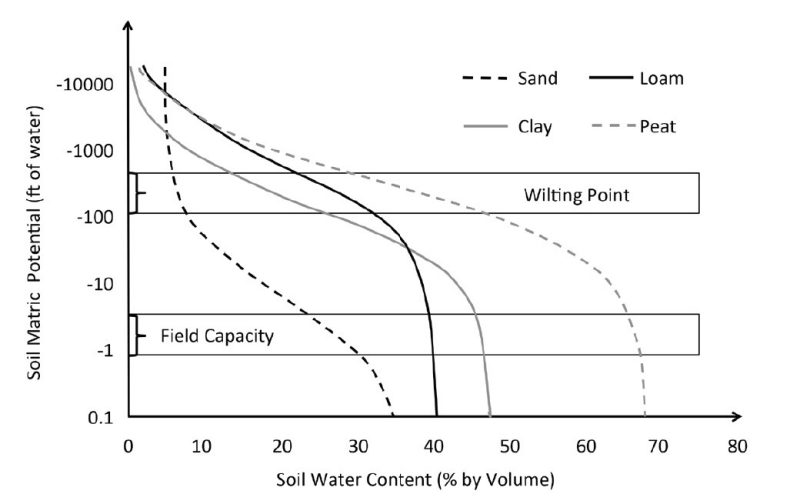
Pressure Potential
Pressure potential is due to the mechanical or hydrostatic force exerted by water in a soil. The pressure potential applies to saturated soils. Pressure potential is the vertical distance between the water surface and a specified point. Pressure potential is zero above the level of water and positive below the water level.
Soil Drainage Classifications
Drainage characteristics are important properties of soils that impact a host of agricultural production properties. Soils that are well-drained tend to be the most productive, provided that rainfall is not limiting, while soils that are poorly drained will restrict root growth and consequently reduce agroecosystem productivity (fig. 8). However, soils that are too well-drained are prone to drought. Figure 8 depicts the five commonly used drainage classifications and how they relate to potential productivity. Very poorly drained soils contain a barrier to root penetration at a shallow depth, between 0 and 18 inches, while well- and moderately well-drained soils are often not depth limited, allowing roots to fully penetrate the soil and thus access available water and nutrients. Soils with restricted drainage often become anaerobic (depleted of oxygen) and reduced (a chemical status favoring certain reactions). Reduced soils commonly have a gray appearance, often with rust colored iron oxide accumulations indicative of a fluctuating water table. This reduced layer can be the result of management (e.g., excessive tillage causing a plow pan) or natural causes (e.g., high water tables, glaciation, fragipan, clay lens) that result in a layer of higher bulk density or restricted drainage.
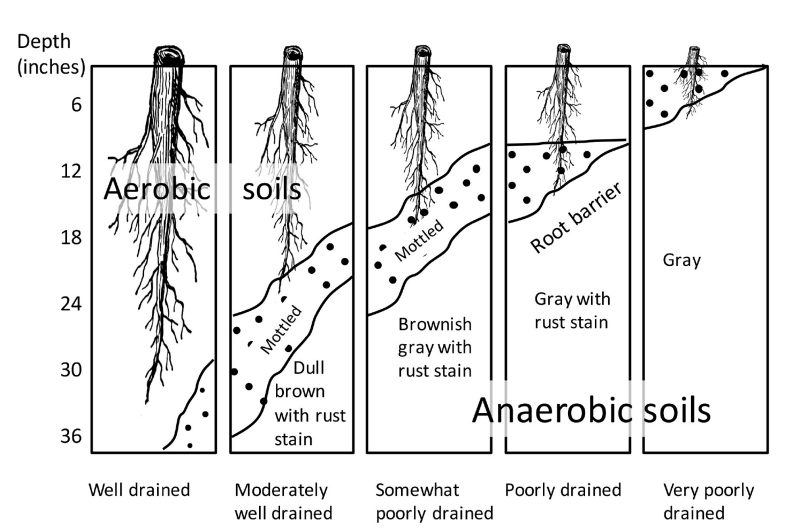
The Water Balance Concept
The water balance, also called a soil water budget, is an important tool that can be used to evaluate past, present, and proposed management practices in an agroecosystem. In its simplest terms, the water balance tracks incoming and outgoing water from a unit such as a field or watershed. The water balance is governed by the basic physical principle of conservation of mass:
inputs − outputs = change in storage.
In this formulation, inputs are precipitation and/ or inflow of ground or surface water, outputs are evapotranspiration and/or outflow of ground or surface water, and change in storage is the change in the moisture content of the unit (field or watershed).
Tracking or predicting soil moisture levels is critical to making management decisions to maximize crop production. The water balance method offers a simple means to calculate a soil water budget for a given field or watershed by using the above formulation and adding a soil water accounting function:
ΔSW = P − AET − QS − QG ,
where ΔSW is the change in soil water from the previous day or month, P is precipitation, AET is actual evapotranspiration, QS is runoff leaving the soil surface or watershed, and QG is the groundwater (percolation) leaving the root zone or watershed. At the watershed scale, QS and QG are often considered together and called streamflow, because groundwater often contributes to streamflow and is difficult to measure in isolation, while measuring streamflow is relatively easy. If precipitation is greater than the sum of actual evapotranspiration, runoff, and percolation/groundwater discharge, the soil is wetting. If precipitation is less than the sum of actual evapotranspiration, runoff, and percolation/ groundwater discharge, the soil is drying.
Figure 9 shows a monthly water balance for the Piedmont region of Virginia. This water balance shows that during the summer growing season, the soil is, on average, drying out due largely to plants removing soil water by transpiration. During the late fall through spring, the soil is generally wetting because plants are not removing as much soil water via transpiration. This is also the time of year when the majority of runoff and groundwater recharge occurs.
The water budget analysis can be performed on a daily basis as well, provided that daily estimates of precipitation and evapotranspiration are available. This analysis could be modified to incorporate future estimates of precipitation and evapotranspiration to develop a short-term forecast water balance, which would notify producers of any predicted droughts or saturated conditions that might impact production. Long-term climate projections that include estimates of change to precipitation and evapotranspiration could likewise be incorporated into a water balance and provide information that could be used to modify planting and harvesting schedules to better utilize soil moisture.
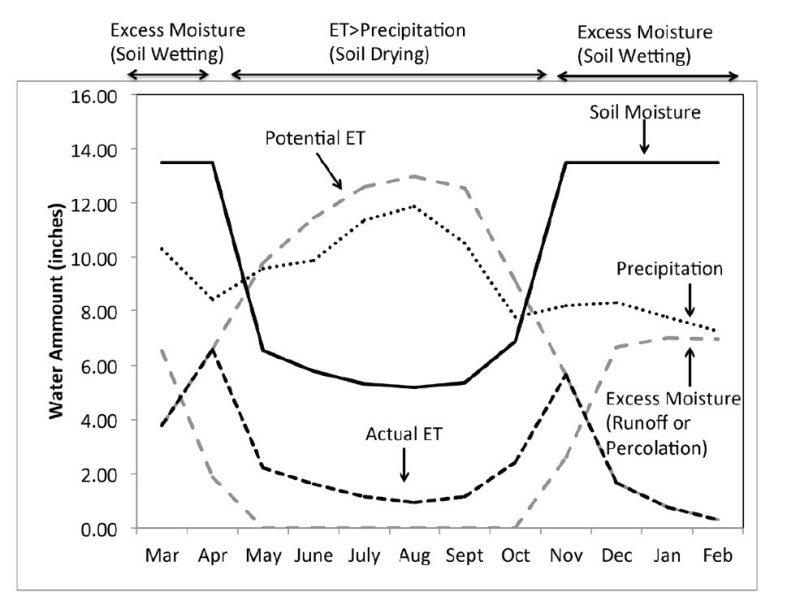
Summary
This publication presents an overview of the basic soil water relationships and highlights some concepts of importance in agroecosystems. We have discussed important properties of soils (composition, texture, structure), how to describe and measure some of these properties (bulk density, porosity, soil water potential, soil drainage class), and how these foundational concepts are applied to manage agricultural systems for optimal productivity. Sound decisions about the use and protection of our water resources require a fundamental understanding of the basic processes controlling soil water movement and cycles. The water balance concept provides a useful tool that can be used to predict field conditions to schedule irrigation or determine the likelihood of leaching that could reduce fertilizer uptake efficiency of crops. Understanding these relationships is critical to marinating and enhancing agricultural productivity and food security.
Additional Resources
USDA Natural Resources Conservation Service: Soil Textural Calculator - www.nrcs.usda.gov/wps/portal/nrcs/detail/soils/survey/?cid=nrcs142p2_054167
USDA Natural Resources Conservation Service: Web Soil Survey - http://websoilsurvey.sc.egov.usda.gov/App/HomePage.htm
Related Virginia Cooperative Extension Publications
Benham, B. 2009. A Glossary of Water-Related Terms. VCE publication 442-758.
Easton, Z. M., and E. M Bock. 2015. Hydrology Basics and the Hydrologic Cycle. VCE publication BSE-191.
Ross, B. 2009. Virginia Farmstead Assessment System: Site Evaluation: Groundwater, Soils & Geology. VCE publication 422-901.
Glossary of Terms
actual evapotranspiration - The quantity of water that is actually removed from the soil due to evaporation and transpiration. AET can be measured in the field via weighting lysimeters or, as is more often the case, at the larger scale (watershed), it is calculated using an equation such as Penman-Monteith or Hargreaves methods.
adhesion - The attraction between water and a soil particle. A drop of water sticks (adheres) to a soil particle.
capillary water distribution - The movement of water up a small pore (capillary) against gravity, due to the attraction of water to the solid surface (adhesion) and the surface tension of water (due to cohesion between water molecules).
cation exchange capacity - The total of exchangeable, positively charged ions (cations) that a soil can absorb. CEC influences the soil’s ability to hold onto essential nutrients and provides a buffer against soil acidification.
cohesion - The attraction of water molecules to each other. Cohesion causes water droplets to form.
conservation of mass - States that matter (in this case, water [H2O]) can change forms (gas, liquid, solid) but that the total mass of water remains constant, that is, the sum of all the mass of liquid, solid, and gas water is always the same.
dispersion - The separation of soil into single particles.
evapotranspiration - The sum of evaporation from the land and water surface and plant transpiration to the atmosphere.
hydrostatic force - The pressure exerted by water at equilibrium due to the force of gravity. Hydrostatic force increases in proportion to depth measured from the surface because of the increasing weight of water exerting downward force from above.
infiltration - The downward entry and movement of water through the soil profile.
macropores - Large soil pores that form as a result of biological activity (root channels, worm holes), geological forces (subsurface erosion, desiccation, and syneresis cracks and fractures), or mechanical practices (plowing, bores, and wells). Macropores are larger than 0.08 mm in diameter and can conduct large quantities of water and agrochemical deeply onto the soil.
micropores - Soil pores smaller than or equal to 0.08 mm inside soil aggregates that retain water and agrochemicals and are responsible for capillary water distribution.
percolation - The downward movement of water through saturated soil layers. Percolation is responsible for groundwater (aquifer) recharge.
soil organic matter - Plant and animal residues, cells and tissues, or soil organisms composed of carbon and the substances the organisms synthesize.
soil texture - The relative proportions of sand, silt, and clay that make up a soil. Many soil properties are influenced by texture, including drainage, water- holding capacity, aeration, susceptibility to erosion, organic matter content, cation exchange capacity, pH buffering capacity, and soil tilth. The combined fractions of sand, silt, and clay in a soil determine its textural classification. Sand particles range in size from 0.05 mm to 2.0 mm, silt ranges from 0.002 mm to 0.05 mm, and the clay fraction is made up of particles smaller than 0.002 mm in diameter. Once the sand, silt, and clay fractions are known, the textural class can be read from the textural triangle (www.nrcs.usda.gov/wps/portal/nrcs/detail/soils/survey/?cid=nrcs142p2_054167).
soil water content - Amount of water a given soil can store, primarily influenced by the soil texture and the soil organic matter content. In general, soils with greater silt and clay-sized particles have greater water- holding capacities. Likewise, soils with more organic matter have greater water-holding capacities.
surface tension - The combined effect of adhesive and cohesive forces at the soil water interface.
tilth - Quality of a soil for purpose of cultivation related to soil structure, including characteristics supporting plant growth (utility as seedbed, obstruction to seedling emergence and root penetration) and the ease of tillage.
water balance - A means to track the volume of water moving in and out of a soil or watershed based on the principle of the conservation of mass.
Virginia Cooperative Extension materials are available for public use, reprint, or citation without further permission, provided the use includes credit to the author and to Virginia Cooperative Extension, Virginia Tech, and Virginia State University.
Virginia Cooperative Extension is a partnership of Virginia Tech, Virginia State University, the U.S. Department of Agriculture (USDA), and local governments, and is an equal opportunity employer. For the full non-discrimination statement, please visit ext.vt.edu/accessibility.
Publication Date
March 1, 2021